Very good summary. I'm gonna borrow some of your analagies in the radiological hazard classes I teach. :-) You might be interested in my Geiger-Müler measurements after Fukushima.
http://www.ecoreality.org/wiki/Radiation_log
I have some background in metrology, and the methodology may be of interest. Bottom line: you need long counts and long samples. The proper way to do this would be capture a stream and filter it, then read the filter. I alternated outdoor readings with indoor readings, and was puzzled when the indoor readings were higher!http://www.ecoreality.org/wiki/Radiation_fallout_plan
I then discovered that my supposed "control" measurements were actually measuring radiation from some dust on old books! Also perhaps of interest is our radiation fallout plan. As Chris points out, the goal is to avoid ingesting contamination.
If Fukushima has taught us anything, its that nuclear power is totally dependent on a functioning electrical grid. A Carrington Event could result in hundreds of Fukushimas around the globe!
http://news.nationalgeographic.com/news/2011/03/110302-solar-flares-sun-storms-earth-danger-carrington-event-science/
==============================================================
FROM
http://www.peakprosperity.com/insider/84663/contamination-threat

Yuliyan Velchev/Shutterstock
Fukushima's Legacy: Understanding the Difference Between Nuclear Radiation & Contamination
It's very important
Tuesday, February 11, 2014, 9:55 AM
alpha, alpha radiation, beta, beta radiation, Contamination, dose, electron, energy, fukushima, gamma, gamma radiation, gamma rays, Gray, ion, Japan, Neutrons, Nuclear, particle, proton, rad, radiation, Reactor, rem, Sievert, tepco, x-ray
Are fish from the Pacific safe to eat? What about the elevated background radiation readings detected in Japan, and recently, in California? Are these harmful levels?
Should we be worried? And if so, what should be done about these potential health threats? What steps should we take to protect ourselves?
As many of you know, I'm a scientist by training. In this report, I'll lay out the facts and data that explain the actual risks. I'll start by pointing out that Fukushima-related fears have been overblown as well as heavily downplayed by parties on each side of the discussion.
Much of this stems from ignorance of the underlying science. But some of it, sadly, seems to be purposefully misleading. Again, on both sides.
To assess the true risks accurately, you need to know about the difference between radiation and contamination. The distinction is vital, and, unfortunately, one of the most glossed-over and misused facets of the reporting on nuclear energy.
While the background levels are elevated somewhat, those detected so far remain well within what I consider to be a safe zone. However, should there be another accident at the damaged facility leading to the release of another large plume of radioactive matter, then this assessment could, understandably, change.
The exception to this assessment is for those living within a hundred kilometers of Fukushima. For those people, my analysis points to serious risks, especially for those living with a kilometer or two of the coast, extending 100 kilometers in either direction. The details behind my assessment are contained in the full report below.
The intent of this report is to help readers understand the likely implications of the Fukushima situation with more clarity, as well as to provide a useful framework for identifying the risks posed by any future nuclear incidents and what your response to them should be.
The most important takeaway from this analysis should be this: Radiation, itself, is less a threat than most people imagine. But radioactive contamination is an entirely different and far more dangerous beast.
While both deliver a ‘dose’ of radiation, it's contamination – especially ingested contamination – that has the greatest odds of delivering a concentrated dose to human tissue in a way that can lead to serious acute and/or chronic damage.
The difference between these two will be explained in detail. For those who chose not to read the full report and just want the punchline, it's this: Contamination is the process of acquiring radioactive particles that then become lodged on, or more dangerously in, your body. Do all you can to protect yourself against it.
Should you find yourself nearby during a nuclear accident, your first order of business is to avoid breathing or ingesting any contaminated particulate matter. This usually involves sheltering in place and is when duct tape and plastic sheeting become your best friends. While it may sound silly to use such a dime-store defense against a nuclear hazard, it is in fact both remarkably effective and entirely necessary. Merely keeping you and your family away from the fallout for a matter of 2-3 days, possibly a bit longer depending on conditions, can make an enormous difference in your survival odds.
For now, the levels of radiation that have been detected and reported outside of Japan are between two and three orders of magnitude below what I would personally consider to be worrisome. And there’s no concrete evidence that the bigger concern, contamination, has traveled to countries outside of Japan.
And within Japan, the story takes on its own complexity (just as happened in the areas surrounding Chernobyl), where local wind patterns in the days after the accident created a complex quilt of danger and (relative) safety.
For those who wish to engage with the context and details of the post-Fukushima world, the journey begins by understanding what ‘radiation’ actually is.
You are bathed in radiation every day: from sunlight, radio waves, wi-fi, etc. Some radiation is electromagnetic (in the case of light), and some is composed of particles (matter).
When we hear about ‘radiation’ in the press, what’s typically being referred to are potentially harmful forms of energetic emissions, both electromagnetic and particulate, that can damage biological organisms.
The main distinction between harmful and benign radiation lies in the ability of the radioactive wave or particle to ionize a molecule in your body. Technically, 'ionizing' means "to create an ion," which involves forcibly stripping an electron off a molecule or atom. This leaves the molecule or atom in a charged state (referred to as 'ionic form'), which thus can cause the affected particle to break apart or otherwise not work as it did before.
For example, the hemoglobin in your blood is a very complex molecule. Breaking even one of its internal bonds can completely destroy its ability to carry oxygen.
Every cell in your body is an enormously complex machine with thousands of different molecules each with a crucial function. Wreck enough of these molecules through the process of ionization and the cell dies. Destroy or disrupt the DNA at the center of the cell, and malfunction will result, one dramatic form being the loss of the ability to self-regulate its growth, which we call cancer.
Radioactive substances emit various forms of energy. Some of the energetic releases are in the form of photon waves (such as gamma or X-rays) while some are in the form of actual fast-moving particles (such as alpha and beta particles, and neutrons).
We lump them all together and call them ‘radiation’. But when it comes to their impact on living organisms, not all forms of radiation are created equally. Some are far more effective 'disrupters of life' than others.
The basic types of radiation you would encounter as a consequence of a nuclear accident like Fukushima are:
To help clarify this, imagine that we're talking about radiation as if it were vehicles traveling on a highway. It's not really possible to predict how destructive it would be to collide with 'a vehicle,' because that answer depends on knowing factors like the vehicle’s size, weight, and speed.
Bumping into a small car traveling slowly in your same direction will be far less damaging than slamming head-on into a large fully-loaded Mack truck going 80 mph.
The way this is technically measured is by the energy that each type of radiation carries, measured in units called 'electron volts' (eV). Think of the eV rating as combining both the speed and the mass of the vehicle we are trying to rank.
To the eV designation, we'll add the scientific shorthand of K for 'kilo' signifying 1,000 and M for Mega signifying 1,000,000. So 1 KeV = 1,000 eV, and 1 MeV = 1,000,000 eV
Along our radiation 'highway,' we find that x-rays carry the least energy and are in the vicinity of 1.2 KeV. They are small, light cars. Think Fiat.
Gamma rays are not a single vehicle type, because they can have energies anywhere from a few KeV all the way up to 25 MeV. They are everything and anything from tiny TR-6s to massive, fully loaded, Peterbilt double trailer trucks traveling 80 mph. For reference, the gamma rays emitted by gesium-137, a very common byproduct of nuclear reactors and a main component of the Fukushima releases, is 700 KeV, hundreds of times more energetic than your typical dentist x-ray, but not nearly the most potent gamma ray you could encounter.
Some common gamma emitters are cesium-137, cobalt-60 and technetium-99. Also, about 10% of the radioactivity of iodine-131 is gamma, the rest is beta (making this is a mixed radioelement).
Alpha particles have very high kinetic energies standing at about 5 MeV. However, they have exceptionally poor penetrating power, so we might think of them as very large steamrollers that can lurch forwards violently, but only for a few feet. If you are right next to it, you're in big trouble, but otherwise you're safe.
In recent years, a potent alpha emitter, polonium-210, was used to assassinate both Yasser Arafat and Russian critic Alexander Litvinenko. Because polonium-210 only emits alpha particles, you could carry it in a glass vial in your pocket and slip though radiation detectors at any facility because none of the alpha particles would make it through the vial wall (and even if they somehow did, they’d be stopped by the fabric of your pants pocket). In fact, you could merrily rub it on your skin and suffer no ill effects.
But if ingested? Just a few milligrams, a speck the size of a small grain of salt, would be sufficient to kill. All those gigantic lurching steamrollers would be positioned right next to your living cells, crashing into them and destroying your tissues one cell at a time.
Common alpha emitters include radium, radon, polonium, uranium, and thorium.
Beta particles are electrons ejected during proton decay, and they travel at high speed. They can range anywhere between 5 KeV and 20 MeV. For our purposes, the isotopes most commonly associated with nuclear reactions are in the range of 19 KeV (tritium) to 600 KeV (iodine-131 and strontium-90) to 2.3 MeV (yttrium-90). So these range from medium-sized cars to tractor-trailers, in our analogy.
Beta particles have medium penetrating power and they can easily get through your skin to the living tissues beneath. Think of them as being able to give you a very harsh sunburn from the outside inwards if you were exposed long enough. Again, their worst effects come if ingested, where they can cause lots of damage.
Some common beta emitters are strontium-90, yttrium-90, iodine-131, carbon-14, and tritium.
Neutrons are a very wide topic, so we'll just talk about them in terms of a nuclear reactor. The moderate to fast neutrons emitted as a product of fission are extraordinarily dangerous and can penetrate lead shields and many meters of concrete. They are most readily stopped by interacting with hydrogen, so water and wax (and human bodies) – which contain lots of hydrogen atoms – are better at stopping neutrons than concrete.
Neutrons are not part of the radioactive release from Fukushima. They really aren't ever an issue unless you somehow find yourself near an open, uncontained source of fission – like inside the containment shell of an operating reactor, or in the vicinity of an exploding nuclear bomb. Then neutrons are a BIG problem.
Of note: In the early stages of the Fukushima meltdown, neutron 'beams' were detected 13 times from outside the reactors. This understandably caused the TEPCO workers a lot of worry and slowed their response efforts. This was a certain indication that there was spontaneous fission happening outside of a sealed containment vessel, something that TEPCO was busily assuring the world had not happened. They were still claiming that the vessels were intact and full of pumped cooling water.
The bottom line is that the topic of radioactivity is complex. If we want to make intelligent decisions, then we need to know which type of radiation we are talking about.
For example, there are folks walking about with mail-order radiation detectors and reporting ‘counts per minute’ readings. But counts of what, exactly? Is each ‘count’ a low-energy beta particle or a high-energy gamma ray? There’s a world of difference between the two.
So we owe it to ourselves to dig into the context before coming to conclusions. To determine how concerned we should be about any new data, we have to translate ‘counts’ of any particle into their potential health effects.
So radiation alone is not a cause for concern for me. Even temporary radiation levels that are significantly above my normal background baseline, as much as ten or twenty times, are not a concern of mine as a healthy adult.
But as our vehicle analogy above showed, first we have to know what kind of radiation we are talking about. Is it alpha, beta, or gamma? How much energy is it carrying?
We also need to know about the person being exposed to the radiation. Tolerance levels for what's "safe" will be lower for kids, the old, and the frail.
For these reasons, science has struggled to come up with a universal measurement for the health impact caused by radiation. As a result, we have several different measurement methodologies parked into a few slightly different, but essentially related, scales. Each attempts to combine the acute effects of radiation exposure into a single 'dose' that is a measure of both the intensity and the duration of the exposure.
As mentioned previously, some radiation has the ability to travel right through our bodies entirely without being absorbed. So, the ‘dose’ reading needs to focus on the amount of any specific radiation type that will be absorbed (or stopped) by the body and thereby have opportunity to impact the molecules in that body.
The radiation absorbed dose is measured in Gray, rad, rem, and Sievert.
Rads and Grays are related to each other. One Gray is a huge dose, and the rad just breaks the Grays down into finer units. One Gray = 100 rads (rad stands for Radiation Absorbed Dose). These measure the amount of energy that ionizing radiation imparts to matter. This matter could be anything: a block of cement, or a human.
Sieverts and rems are likewise related. One Sievert = 100 rems, but these are adjusted to provide a measure of the impact of the absorbed dose of ionizing radiation on biological tissue. To equate the two systems, the absorbed dose in Grays or rads is multiplied by a 'quality factor' that is specific to each type of radiation to account for their different biological impacts: the result is Sieverts or rems. Thus, using our vehicle analogy from before, our small sedans get an adjustment factor of 1, while heavier vehicles get an adjustment factor as high as 10-20 times greater.
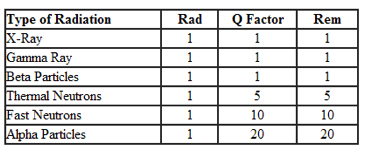
Keep in mind this table is a huge simplification of a very complicated field of study. For example, it also matters which tissues are being exposed, as they have very different sensitivities to radiation.
However, if we are talking about an episode of external exposure to radiation, like a worker at Fukushima might get, then we care about the Sievert or rem scale:
Thus, dose is a function of intensity and time. You may recall seeing the grainy footage of Chernobyl ‘workers’ ducking out from behind cover and racing to move a single wheelbarrow of rubble from point A to point B. In those few seconds, they may have received a lifetime maximum dose of radiation and were (hopefully) sent home after accomplishing that one task.
The average global background radiation is 0.27 microS/hour (that's millionths of a Sievert). If we multiply that number by 24x365, it yields an average yearly dose of 2.4 mS/yr. TEPCO workers are permitted to receive 250 mS/yr, while U.S. nuclear worker standards are 100 mS/yr, which is roughly 25 times greater than background.
The average airport security screening device delivers a dose of 0.25 microS, or the equivalent of a full day's background radiation. If that alarms you, just know that during the actual flight you take, the average exposure is ten times higher than that – providing 2.7 microS per hour of flight at cruising altitude, or ten times normal background. So a 5-hour flight at cruising altitude will provide you with a dose of gamma radiation that measures 54 times more than you get at the airport screening itself, or two full days worth of background radiation.
Again, at these levels, I am not even remotely concerned. If there were something to worry about, then the epidemiological data from flight attendants and pilots would have long ago revealed a health concern. That's one reason why I'm not worried about periodic episodes of 10x normal background radiation.
Of course, the Sievert is a very crude scale, developed a long time ago. One might argue that the biological impact of airport screeners and whole-body gamma irradiation might be more subtle and complex due to differences in tissue responses and how the radiation is concentrated on the surface of the skin by airport scanners. All of that remains an open question to me, but not enough of one to concern me.
Still, the point here is that we are surrounded by radiation all the time, and we absorb a yearly dose no matter where we live – but Denver-ites get a lot more than people living in Miami due to the altitude (less atmospheric protection from extra planetary gamma arrays).
Here's a link to a super useful graphic that visually shows the Sievert doses of both ordinary life and the Fukushima accident in relation to each other.
Based on this chart, plus all of the information above, even if your background radiation goes up by a factor of ten or twenty, I wouldn't be concerned.
By "contamination," I mean ingesting some radioactive isotopes or particles that become lodged in the body somehow. Perhaps it's a small speck of radioactive dust that gets lodged in the lung where it will persist (like coal dust and asbestos do), or perhaps it's a substance that our bodies try to accumulate because it resembles a biologically useful element (as is the case with iodine or strontium).
In Part II: The Contamination Threat, we examine in depth the threats posed by radioactive contamination, including the most prevalent contaminants to be wary of, and the compounding effects of bioaccumulation and biomagnification. One of the most nefarious aspects of contamination is how it uses Nature's processes against itself.
For the record, we are aware of no imminent public health threat from nuclear contamination outside of already-identified "hot zones." But for those who wish to better understand the risks and prudent protection measures related to the real dangers of a similar Fukushima-type event in the future (or an unfortunate escalation of the current Fukushima situation), being forewarned is forearmed.
Click here to access Part II of this report (free executive summary; enrollment required for full access).
The much-feared plutonium-238 is also an alpha emitter. On the outside of your body it is not much of a problem. Inside it is extraordinarily harmful.
Iodine-131 (I-131) is another "fairly harmless on the outside, but deadly on the inside" sort of substance. Even a vastly sub-lethal dose of I-131 in terms of your whole body load will be damaging if not deadly, because iodine is viewed by your body as a delicious and rare treat, with your thyroid gobbling it up, radioactive or not, and storing it for future use.
As the thyroid does this, the I-131 gets concentrated into a very small body mass where the radioactive load experienced by the thyroid is far higher than any surrounding tissues. At a high enough dose, the thyroid will be destroyed which is a survivable experience, as anybody who's had their thyroid removed can attest.
The real difficulty actually comes with...
Should we be worried? And if so, what should be done about these potential health threats? What steps should we take to protect ourselves?
As many of you know, I'm a scientist by training. In this report, I'll lay out the facts and data that explain the actual risks. I'll start by pointing out that Fukushima-related fears have been overblown as well as heavily downplayed by parties on each side of the discussion.
Much of this stems from ignorance of the underlying science. But some of it, sadly, seems to be purposefully misleading. Again, on both sides.
To assess the true risks accurately, you need to know about the difference between radiation and contamination. The distinction is vital, and, unfortunately, one of the most glossed-over and misused facets of the reporting on nuclear energy.
Starting with the Bottom Line
All of my research and understanding of the risks of radiation at this point indicate that people living on the West Coast of the U.S. or in Hawaii are currently not in danger from the radiation released in the wake of the Fukushima tragedy.While the background levels are elevated somewhat, those detected so far remain well within what I consider to be a safe zone. However, should there be another accident at the damaged facility leading to the release of another large plume of radioactive matter, then this assessment could, understandably, change.
The exception to this assessment is for those living within a hundred kilometers of Fukushima. For those people, my analysis points to serious risks, especially for those living with a kilometer or two of the coast, extending 100 kilometers in either direction. The details behind my assessment are contained in the full report below.
The intent of this report is to help readers understand the likely implications of the Fukushima situation with more clarity, as well as to provide a useful framework for identifying the risks posed by any future nuclear incidents and what your response to them should be.
The most important takeaway from this analysis should be this: Radiation, itself, is less a threat than most people imagine. But radioactive contamination is an entirely different and far more dangerous beast.
While both deliver a ‘dose’ of radiation, it's contamination – especially ingested contamination – that has the greatest odds of delivering a concentrated dose to human tissue in a way that can lead to serious acute and/or chronic damage.
The difference between these two will be explained in detail. For those who chose not to read the full report and just want the punchline, it's this: Contamination is the process of acquiring radioactive particles that then become lodged on, or more dangerously in, your body. Do all you can to protect yourself against it.
Should you find yourself nearby during a nuclear accident, your first order of business is to avoid breathing or ingesting any contaminated particulate matter. This usually involves sheltering in place and is when duct tape and plastic sheeting become your best friends. While it may sound silly to use such a dime-store defense against a nuclear hazard, it is in fact both remarkably effective and entirely necessary. Merely keeping you and your family away from the fallout for a matter of 2-3 days, possibly a bit longer depending on conditions, can make an enormous difference in your survival odds.
For now, the levels of radiation that have been detected and reported outside of Japan are between two and three orders of magnitude below what I would personally consider to be worrisome. And there’s no concrete evidence that the bigger concern, contamination, has traveled to countries outside of Japan.
And within Japan, the story takes on its own complexity (just as happened in the areas surrounding Chernobyl), where local wind patterns in the days after the accident created a complex quilt of danger and (relative) safety.
For those who wish to engage with the context and details of the post-Fukushima world, the journey begins by understanding what ‘radiation’ actually is.
Radiation Types
What do we mean when we say 'radiation'? As it turns out, that word can mean any number of things.You are bathed in radiation every day: from sunlight, radio waves, wi-fi, etc. Some radiation is electromagnetic (in the case of light), and some is composed of particles (matter).
When we hear about ‘radiation’ in the press, what’s typically being referred to are potentially harmful forms of energetic emissions, both electromagnetic and particulate, that can damage biological organisms.
The main distinction between harmful and benign radiation lies in the ability of the radioactive wave or particle to ionize a molecule in your body. Technically, 'ionizing' means "to create an ion," which involves forcibly stripping an electron off a molecule or atom. This leaves the molecule or atom in a charged state (referred to as 'ionic form'), which thus can cause the affected particle to break apart or otherwise not work as it did before.
For example, the hemoglobin in your blood is a very complex molecule. Breaking even one of its internal bonds can completely destroy its ability to carry oxygen.
Every cell in your body is an enormously complex machine with thousands of different molecules each with a crucial function. Wreck enough of these molecules through the process of ionization and the cell dies. Destroy or disrupt the DNA at the center of the cell, and malfunction will result, one dramatic form being the loss of the ability to self-regulate its growth, which we call cancer.
Radioactive substances emit various forms of energy. Some of the energetic releases are in the form of photon waves (such as gamma or X-rays) while some are in the form of actual fast-moving particles (such as alpha and beta particles, and neutrons).
We lump them all together and call them ‘radiation’. But when it comes to their impact on living organisms, not all forms of radiation are created equally. Some are far more effective 'disrupters of life' than others.
The basic types of radiation you would encounter as a consequence of a nuclear accident like Fukushima are:
- Alpha particles. These are fast moving nuclei of helium, meaning that they consist of two protons and two neutrons. The electron shell is missing, so these are charged particles in search of electrons to strip from some other hapless molecule or atom. In the subatomic world, these are very large particles and so are the most easily stopped. They cannot penetrate even a single sheet of paper or the layer of dead skin cells on the outside of your body. As a result, they are quite easy to protect against with minimal effort. However, we shouldn't take total comfort in this fact. The deadly toxin polonium-210, the one used to kill various enemies of the Russians over the years, emits alpha particles and is quite effective as a poison. The reason for this lies in the fact that, once ingested, it works its damage in close proximity to a person's cells. On the outside of a body, alpha particles bump into already-dead skin cells, so no harmful damage results. On the inside, they careen straight into living cells and are quite damaging.
- Beta particles. These are electrons that have been ejected through a radioactive decay process (technically, it's when a neutron decays, yielding both a proton and an electron). Beta radiation can penetrate a sheet of paper easily, and it requires something along the lines of an aluminum plate a few millimeters thick to stop it. Beta particles have medium ionizing power and medium penetrating power, but there is a very wide spectrum of potential power intensities, depending on exactly which radioactive substance is emitting the beta particle. One very common radioactive substance found in nuclear plants, tritium, is a beta emitter.
- Gamma rays. These are high-energy photons with strong penetrating power and high ionizing potential. In the past, they were distinguished from x-rays on the basis of their energy potential, but they are really the same thing (they are both high-energy photons). Although, what we call an x-ray generally carries a lot less energy than a gamma ray. That is, an x-ray is at the low end of the energetic spectrum, while a gamma ray is at the higher end. This is exactly analogous to the difference between visible sunlight and UV rays, which are the radiation (composed of high-energy photons) that burns your skin. Just place gamma rays a lot further along that same spectrum all the way at the point where, instead of being stopped by your underlay of skin, the gamma rays can create an equivalent ‘sunburn’ on tissues all the way through your body. Gamma rays vary in strength and actually occupy a spectrum of energies (not unlike how white light includes the spectrum of all the colors of the rainbow), so we need to know more about the specific gamma rays in question to know how damaging they might be.
- Neutrons. Neutrons are the bad boys of the radiation story, and are only found as a consequence of a nuclear reaction (controlled or uncontrolled). Their penetrating power is extraordinary, requiring several meters of solid substance to stop them. They work their harm by indirect ionization, which is not unlike a pool ball smashing into a lamp. A typical example would be the capture of a neutron by a hydrogen nucleus consisting of a single proton, which is then ripped away from its position by the kinetic energy contained by the neutron, and then, like our billiard ball, careens about breaking things, ionizing some atoms/molecules, or shattering the bonds between atoms. In terms of biological damage, neutrons are horrific – roughly ten times more damaging than beta or gamma radiation on a per-unit-of-energy basis.
To help clarify this, imagine that we're talking about radiation as if it were vehicles traveling on a highway. It's not really possible to predict how destructive it would be to collide with 'a vehicle,' because that answer depends on knowing factors like the vehicle’s size, weight, and speed.
Bumping into a small car traveling slowly in your same direction will be far less damaging than slamming head-on into a large fully-loaded Mack truck going 80 mph.
The way this is technically measured is by the energy that each type of radiation carries, measured in units called 'electron volts' (eV). Think of the eV rating as combining both the speed and the mass of the vehicle we are trying to rank.
To the eV designation, we'll add the scientific shorthand of K for 'kilo' signifying 1,000 and M for Mega signifying 1,000,000. So 1 KeV = 1,000 eV, and 1 MeV = 1,000,000 eV
Along our radiation 'highway,' we find that x-rays carry the least energy and are in the vicinity of 1.2 KeV. They are small, light cars. Think Fiat.
Gamma rays are not a single vehicle type, because they can have energies anywhere from a few KeV all the way up to 25 MeV. They are everything and anything from tiny TR-6s to massive, fully loaded, Peterbilt double trailer trucks traveling 80 mph. For reference, the gamma rays emitted by gesium-137, a very common byproduct of nuclear reactors and a main component of the Fukushima releases, is 700 KeV, hundreds of times more energetic than your typical dentist x-ray, but not nearly the most potent gamma ray you could encounter.
Some common gamma emitters are cesium-137, cobalt-60 and technetium-99. Also, about 10% of the radioactivity of iodine-131 is gamma, the rest is beta (making this is a mixed radioelement).
Alpha particles have very high kinetic energies standing at about 5 MeV. However, they have exceptionally poor penetrating power, so we might think of them as very large steamrollers that can lurch forwards violently, but only for a few feet. If you are right next to it, you're in big trouble, but otherwise you're safe.
In recent years, a potent alpha emitter, polonium-210, was used to assassinate both Yasser Arafat and Russian critic Alexander Litvinenko. Because polonium-210 only emits alpha particles, you could carry it in a glass vial in your pocket and slip though radiation detectors at any facility because none of the alpha particles would make it through the vial wall (and even if they somehow did, they’d be stopped by the fabric of your pants pocket). In fact, you could merrily rub it on your skin and suffer no ill effects.
But if ingested? Just a few milligrams, a speck the size of a small grain of salt, would be sufficient to kill. All those gigantic lurching steamrollers would be positioned right next to your living cells, crashing into them and destroying your tissues one cell at a time.
Common alpha emitters include radium, radon, polonium, uranium, and thorium.
Beta particles are electrons ejected during proton decay, and they travel at high speed. They can range anywhere between 5 KeV and 20 MeV. For our purposes, the isotopes most commonly associated with nuclear reactions are in the range of 19 KeV (tritium) to 600 KeV (iodine-131 and strontium-90) to 2.3 MeV (yttrium-90). So these range from medium-sized cars to tractor-trailers, in our analogy.
Beta particles have medium penetrating power and they can easily get through your skin to the living tissues beneath. Think of them as being able to give you a very harsh sunburn from the outside inwards if you were exposed long enough. Again, their worst effects come if ingested, where they can cause lots of damage.
Some common beta emitters are strontium-90, yttrium-90, iodine-131, carbon-14, and tritium.
Neutrons are a very wide topic, so we'll just talk about them in terms of a nuclear reactor. The moderate to fast neutrons emitted as a product of fission are extraordinarily dangerous and can penetrate lead shields and many meters of concrete. They are most readily stopped by interacting with hydrogen, so water and wax (and human bodies) – which contain lots of hydrogen atoms – are better at stopping neutrons than concrete.
Neutrons are not part of the radioactive release from Fukushima. They really aren't ever an issue unless you somehow find yourself near an open, uncontained source of fission – like inside the containment shell of an operating reactor, or in the vicinity of an exploding nuclear bomb. Then neutrons are a BIG problem.
Of note: In the early stages of the Fukushima meltdown, neutron 'beams' were detected 13 times from outside the reactors. This understandably caused the TEPCO workers a lot of worry and slowed their response efforts. This was a certain indication that there was spontaneous fission happening outside of a sealed containment vessel, something that TEPCO was busily assuring the world had not happened. They were still claiming that the vessels were intact and full of pumped cooling water.
The bottom line is that the topic of radioactivity is complex. If we want to make intelligent decisions, then we need to know which type of radiation we are talking about.
For example, there are folks walking about with mail-order radiation detectors and reporting ‘counts per minute’ readings. But counts of what, exactly? Is each ‘count’ a low-energy beta particle or a high-energy gamma ray? There’s a world of difference between the two.
So we owe it to ourselves to dig into the context before coming to conclusions. To determine how concerned we should be about any new data, we have to translate ‘counts’ of any particle into their potential health effects.
Radiation's Effect on Our Health
Okay, here's the thing most people don't know about radiation: We are surrounded by it and have evolved with it over billions of years. The body can deal with exposure to a certain amount of ionizing radiation without any difficulty at all. Naturally occurring radioactive elements, such as uranium and radon and carbon-14, have been a part of life since the very beginning. Gamma rays rain down from the celestial heavens every day.So radiation alone is not a cause for concern for me. Even temporary radiation levels that are significantly above my normal background baseline, as much as ten or twenty times, are not a concern of mine as a healthy adult.
But as our vehicle analogy above showed, first we have to know what kind of radiation we are talking about. Is it alpha, beta, or gamma? How much energy is it carrying?
We also need to know about the person being exposed to the radiation. Tolerance levels for what's "safe" will be lower for kids, the old, and the frail.
For these reasons, science has struggled to come up with a universal measurement for the health impact caused by radiation. As a result, we have several different measurement methodologies parked into a few slightly different, but essentially related, scales. Each attempts to combine the acute effects of radiation exposure into a single 'dose' that is a measure of both the intensity and the duration of the exposure.
As mentioned previously, some radiation has the ability to travel right through our bodies entirely without being absorbed. So, the ‘dose’ reading needs to focus on the amount of any specific radiation type that will be absorbed (or stopped) by the body and thereby have opportunity to impact the molecules in that body.
The radiation absorbed dose is measured in Gray, rad, rem, and Sievert.
Rads and Grays are related to each other. One Gray is a huge dose, and the rad just breaks the Grays down into finer units. One Gray = 100 rads (rad stands for Radiation Absorbed Dose). These measure the amount of energy that ionizing radiation imparts to matter. This matter could be anything: a block of cement, or a human.
Sieverts and rems are likewise related. One Sievert = 100 rems, but these are adjusted to provide a measure of the impact of the absorbed dose of ionizing radiation on biological tissue. To equate the two systems, the absorbed dose in Grays or rads is multiplied by a 'quality factor' that is specific to each type of radiation to account for their different biological impacts: the result is Sieverts or rems. Thus, using our vehicle analogy from before, our small sedans get an adjustment factor of 1, while heavier vehicles get an adjustment factor as high as 10-20 times greater.
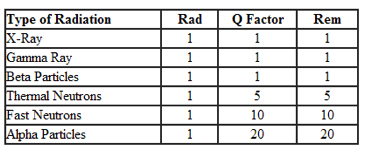
(Source)
Based on this table, it's no wonder that polonium-210 is such a devastating radiological poison, because alpha particle get an adjustment factor of 20 (!), making them twice as deadly as fast neutrons, even. But, again, the alpha particles have to be ingested to have that impact, whereas neutrons can travel through ten feet of concrete and still be dangerous.Keep in mind this table is a huge simplification of a very complicated field of study. For example, it also matters which tissues are being exposed, as they have very different sensitivities to radiation.
However, if we are talking about an episode of external exposure to radiation, like a worker at Fukushima might get, then we care about the Sievert or rem scale:
- 1 Sievert (or 1 Sv), or 100 rem, will induce nausea and reduce the white blood cell count
- 5 Sv, or 500 rems, would cause death for 50% of those exposed in a matter of months
- 10 Sv, or 1,000 rems, is 100% fatal within weeks
Thus, dose is a function of intensity and time. You may recall seeing the grainy footage of Chernobyl ‘workers’ ducking out from behind cover and racing to move a single wheelbarrow of rubble from point A to point B. In those few seconds, they may have received a lifetime maximum dose of radiation and were (hopefully) sent home after accomplishing that one task.
The average global background radiation is 0.27 microS/hour (that's millionths of a Sievert). If we multiply that number by 24x365, it yields an average yearly dose of 2.4 mS/yr. TEPCO workers are permitted to receive 250 mS/yr, while U.S. nuclear worker standards are 100 mS/yr, which is roughly 25 times greater than background.
The average airport security screening device delivers a dose of 0.25 microS, or the equivalent of a full day's background radiation. If that alarms you, just know that during the actual flight you take, the average exposure is ten times higher than that – providing 2.7 microS per hour of flight at cruising altitude, or ten times normal background. So a 5-hour flight at cruising altitude will provide you with a dose of gamma radiation that measures 54 times more than you get at the airport screening itself, or two full days worth of background radiation.
Again, at these levels, I am not even remotely concerned. If there were something to worry about, then the epidemiological data from flight attendants and pilots would have long ago revealed a health concern. That's one reason why I'm not worried about periodic episodes of 10x normal background radiation.
Of course, the Sievert is a very crude scale, developed a long time ago. One might argue that the biological impact of airport screeners and whole-body gamma irradiation might be more subtle and complex due to differences in tissue responses and how the radiation is concentrated on the surface of the skin by airport scanners. All of that remains an open question to me, but not enough of one to concern me.
Still, the point here is that we are surrounded by radiation all the time, and we absorb a yearly dose no matter where we live – but Denver-ites get a lot more than people living in Miami due to the altitude (less atmospheric protection from extra planetary gamma arrays).
Here's a link to a super useful graphic that visually shows the Sievert doses of both ordinary life and the Fukushima accident in relation to each other.
Based on this chart, plus all of the information above, even if your background radiation goes up by a factor of ten or twenty, I wouldn't be concerned.
Contamination Is the Real Danger
But radioactive contamination? That's a whole different beast.By "contamination," I mean ingesting some radioactive isotopes or particles that become lodged in the body somehow. Perhaps it's a small speck of radioactive dust that gets lodged in the lung where it will persist (like coal dust and asbestos do), or perhaps it's a substance that our bodies try to accumulate because it resembles a biologically useful element (as is the case with iodine or strontium).
In Part II: The Contamination Threat, we examine in depth the threats posed by radioactive contamination, including the most prevalent contaminants to be wary of, and the compounding effects of bioaccumulation and biomagnification. One of the most nefarious aspects of contamination is how it uses Nature's processes against itself.
For the record, we are aware of no imminent public health threat from nuclear contamination outside of already-identified "hot zones." But for those who wish to better understand the risks and prudent protection measures related to the real dangers of a similar Fukushima-type event in the future (or an unfortunate escalation of the current Fukushima situation), being forewarned is forearmed.
Click here to access Part II of this report (free executive summary; enrollment required for full access).
Executive Summary
- The "usual suspects" of dangerous radioactive contamination
- Recently reported incidents of contamination
- How bioaccumulation and biomagnification exacerbate the impact of contamination
- Prudent advice post-Fukushima
If you have not yet read Part I: Fukushima's Legacy: Understanding the Difference Between Radiation & Contamination, available free to all readers, please click here to read it first.
Sources of Radioactive Contamination
As mentioned in Part I, polonium provides an excellent and dramatic example of something that is perfectly safe on the outside of the body and perfectly deadly on the inside. That's the difference between radiation and contamination.Yes, polonium-210 is highly radioactive – a half gram of it in a vial will heat itself up to 500 degrees Celsius all on its own just because of radioactive decay – but it is not at all lethal until and unless it is ingested. Once it gets inside, then a fleck the size of a grain of pepper is lethal."Radiation, just like with any toxic chemical, is related to dose," said Cham Dallas, a professor and toxicologist at the University of Georgia's Institute for Health Management and Mass Destruction Defense. "If you get a big dose, then you'll die sooner."And with polonium-210, a dangerous dose can be a matter of micrograms: smaller than a single speck of pepper, he said.If you ingest polonium-210, about 50% to 90% of the substance will exit the body through feces, according to a fact sheet from Argonne National Laboratory. What is left will enter the bloodstream. About 45% of polonium ingested gets into the spleen, kidneys and liver, and 10% is deposited in the bone marrow.Radiation poisoning from polonium-210 looks like the end stage of cancer, Dallas said.Liver and kidney damage ensue, along with extreme nausea and severe headaches. Victims often experience vomiting, diarrhea and hair loss. The alpha particles emitted from the decaying substance get absorbed in the body, which is what causes harm. Death may come in a matter of days, sometimes weeks.
The much-feared plutonium-238 is also an alpha emitter. On the outside of your body it is not much of a problem. Inside it is extraordinarily harmful.
Iodine-131 (I-131) is another "fairly harmless on the outside, but deadly on the inside" sort of substance. Even a vastly sub-lethal dose of I-131 in terms of your whole body load will be damaging if not deadly, because iodine is viewed by your body as a delicious and rare treat, with your thyroid gobbling it up, radioactive or not, and storing it for future use.
As the thyroid does this, the I-131 gets concentrated into a very small body mass where the radioactive load experienced by the thyroid is far higher than any surrounding tissues. At a high enough dose, the thyroid will be destroyed which is a survivable experience, as anybody who's had their thyroid removed can attest.
The real difficulty actually comes with...